Fundamentals of solvents and supporting electrolytes
- Part 1: About solvents
- Part 2: Effect of relative permittivity of the solvent (1)
- Part 3: Effect of relative permittivity of the solvent (2)
- Part 4: Solvent donating and accepting properties and solvent classification
- Part 5: Factors involved in solvation of ions (1)
- Part 6: Factors involved in solvation of ions (2)
- Part 7: Acid-base equilibrium and pH range in organic solvents (1)
- Part 8: Acid-base equilibrium and pH range in organic solvents (2)
- Part 9: Supporting Electrolytes
- Part 10: Potential range of electrochemical measurement (Part 1)
- Part 11: Potential range of electrochemical measurement (Part 2)
- Part 12: The effect of solvents on electrode reactions
- Part 13: Effect of supporting electrolytes
Part 1: About solvents
When we think of solvents, we immediately think of water, and indeed water is a very good solvent. However, there are now many excellent solvents besides water, some of which are widely used in electrode reactions.
These solvents can be divided into 1) molecular solvents consisting mainly of molecules and 2) ionic solvents consisting of anions and cations.
Molecular solvents can be divided into two main categories: aqueous and non-aqueous solvents. Non-aqueous solvents can be further divided into organic solvents and other molecular solvents, such as hydrogen fluoride, liquid ammonia, sulfur dioxide, etc. Solvents other than water are collectively referred to as non-aqueous solvents. Most ionic solvents can be divided into high temperature molten salts, which are electrolytes, and ionic liquids, which have recently received much attention as room temperature liquids.
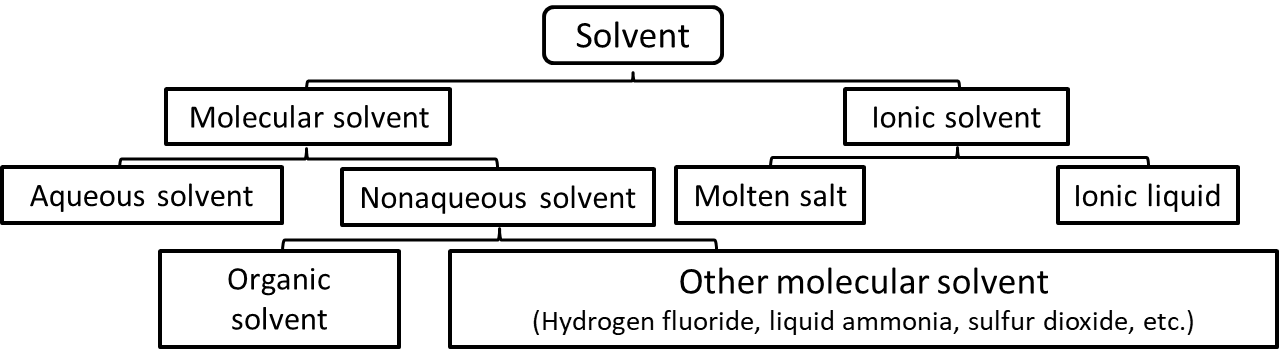
Fig. 1-1 Schematic diagram of solvent types
Water is widely used as an excellent electrode reaction solvent. On the other hand, when suitable solvents other than water are used for electrode reactions, various effects that differ from those of aqueous solutions can be obtained.
For example:
1) Substances insoluble in water can be dissolved.
2) Substances (electrode materials, electrode reactants, intermediates or products, etc.) that are unstable due to reaction with water in aqueous solution can be stabilized.
3) Electrochemical reactions can be measured over a wider potential range, pH range and temperature range than in aqueous solutions.
4) By changing the dissolution state and reactivity of the solute, the reaction mechanism can be made more compatible with the intended application, etc.
The use of non-aqueous solvents for electrochemical experiments is very effective when it is necessary to increase the solubility of the active substance of the electrode or to limit the participation of hydrogen ions in electrochemical reactions and when measurements at low temperature are required to extend the lifetime of the product.
Moreover, the electrochemical method is also the most basic and effective means to analyze the characteristics of the solvent itself and the behavior of the solute in it.
Before discussing solvents for electrode reactions, it is necessary to review the definitions of two physical quantities, the dielectric constant and the relative dielectric constant.
Dielectric constant ε: the constant determined by the response (dielectric polarization) produced by atoms (or molecules) in a substance when an electric field is applied from outside.
Relative permittivity εr: the ratio between the dielectric constant of a medium and the dielectric constant of the vacuum. It is dimensionless quantity.
The relative permittivity εr of the solvent is the most important property affecting the solvation of ions and the dissociation of electrolytes.
Part 2: Effect of relative permittivity of the solvent (1)
The solvation energy of ions is related to several elements of the ion-solvent interaction, where the electrostatic solvation energy ΔGel can be expressed by the following Born formula[1]。
In equation (1), ze is the charge of the ion; r is the radius; N is Avogadro's constant; εr is the relative permittivity. Assuming that r is a constant in this equation, the absolute value of ΔGel decreases as εr decreases. However, the decrease in the absolute value of ΔGel is relatively flat in the range of εr greater than 20, but shows a sharp decrease in the range of εr less than 10. Since the electrostatic solvation energy ΔGel occupies most of the total solvation energy of ions, solvents with small relative dielectric constants (especially εr < 5) generally have weaker solventization of ions due to their electrostatic solvation energy ΔGel becoming smaller in absolute value.
In addition, the cations and anions in solution can exist in a dissociated state that allows the solution to conduct electricity, or they can combine as ion pairs, as shown in equation (2).

Assuming that the constant of the association reaction between the solvated cation and solvated anion is Kass, logKass can be roughly expressed by the following Fuoss approximation equation (3).

where z+e and z-e are the charges of M+ and X- and α is the closest distance between M+ and X- ions .
From equation (3), it can be seen that logKass is almost linearly related to the inverse of αεr, and it can be predicted that if the relative permittivity εr is smaller, or the nearest distance α between ions is smaller, the easier it is to form ion pairs whose solution conductivity is reduced.
The relationship between log Kass and εr inverse for tetrabutylammonium picrate with larger values of α is seen below, and a better linear relationship can be seen between them.
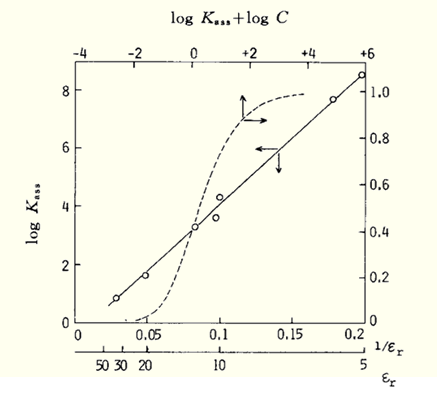
solvents: ➀ C6H5NO2, ➁ Ac, ➂ Py, ➃ CH2ClCH2Cl, ➄ CH3CHCl2, ➅ C6H5Cl, ➆ m-C6H4Cl2
(Y. H. Inami et al., J. Phys. Chem. 83, 4745 (1961).
The dashed line is the relationship between the degree of conformance α and log(C Kass ) (molar concentration of C: Bu4NPic mol dm-3).
Assuming that the analytical concentrations of the tetrabutylammonium cation and picrate anion in solution are C M (M = mol dm-3), there is a relationship between the degree of association α between M+ and X- and log(C Kass ) as shown by the dashed line in Fig. 2-1. For example, for 0.01 M tetrabutylammonium picrate, it can be seen that more than 80% dissociation occurs when the εr of the solvent used is >30, while more than 90% exists as ion pairs when the εr of the solvent is <10.
Reference
[1] A. J. Bard, ed., Electroanalytical Chemistry, Vol. 3, C. K. Mann, Non-aqueous solvents for electrochemical use, p. 57 (1969), Marcel Dekker.
Part 3: Efect of relative permittivity of the solvent (2)
The reaction in which a solid electrolyte is dissolved in a solvent and dissociates into free ions can be divided into two steps: the solid is dissolved in a solvent to form a solvated ion pair (enter↓), and the solvated ion pair is further dissociated to form a solvated cation and a solvated anion.
(M+, X-)solvent ⇔ M+solvent + X-solvent
(5)
For the above two processes, the larger the relative permittivity of the solvent (or other factors that make the solvation of ions easier to occur), the easier it is to move to the right. The solvent used for the electrode reaction should be able to dissolve the supporting electrolyte, and must be able to dissociate into ions to a considerable extent, so a solvent with a relatively large relative permittivity (εr>20) is often used.
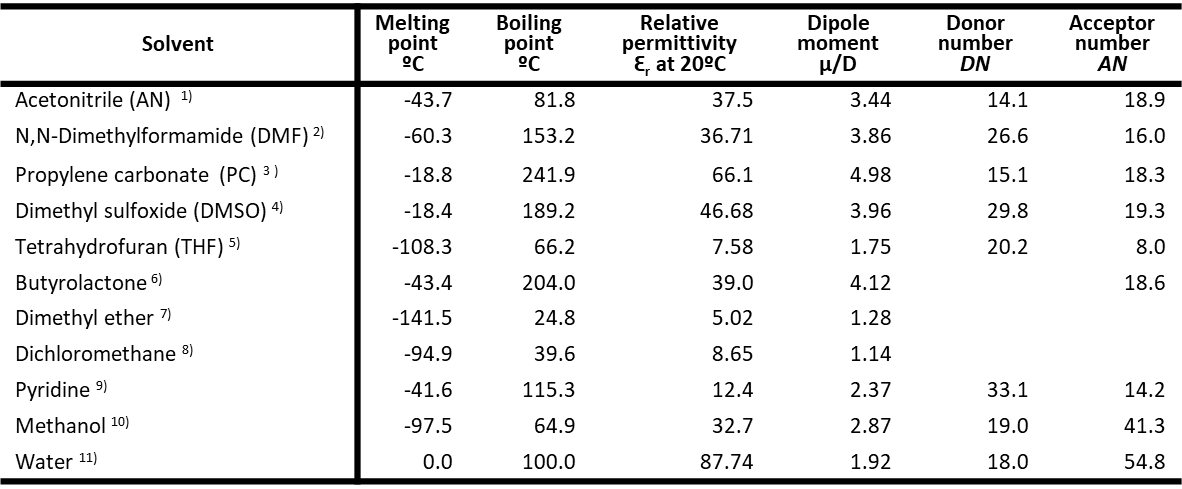

Some typical solvents used in electrochemistry and their physical properties are exemplified in Table 1.
It can be seen that the relative permittivity of solvents commonly used in electrochemistry, such as acetonitrile, DMF, PC, DMSO, methanol, etc., is much higher than 20.
The special mention should be made of the fact that the εr of water is very large, so water is a very good solvent for electrochemical measurements.
The solvents used for electrode reactions should be able to dissolve the supporting electrolyte and must be able to dissociate into ions to a considerable extent, so solvents with large relative dielectric constants (εr > 20) are often used.
However, solvents with low relative dielectric constants, such as THF, can also be used for electrode reaction measurements by using a suitable supporting electrolyte.
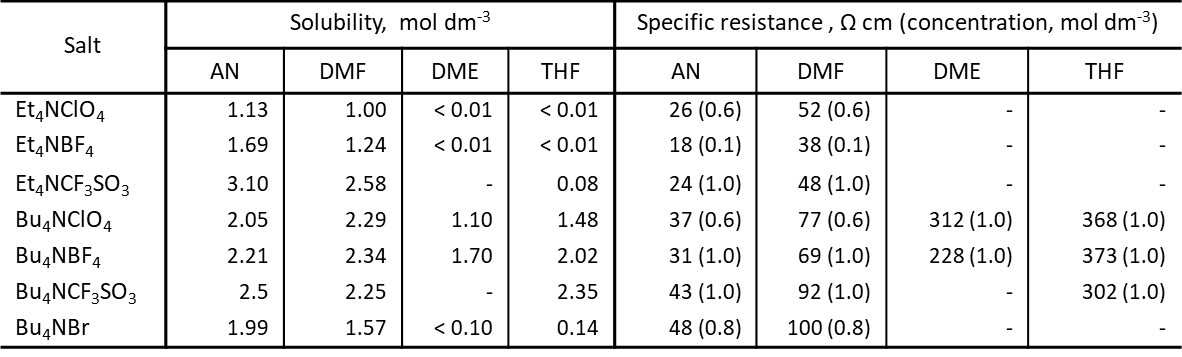
Table 2 shows the solubility and resistivity of various tetraalkylammonium salts in four solvents, acetonitrile, DMF, DME, and THF, at a concentration of 0.6 or 1.0 M.[2][3]
From the solubilities and resistivities in the table, for acetonitrile, DMF, which have relative dielectric constants much greater than 20, the various tetraalkylammonium salts are well dissolved in the solvents, yielding electrolyte solutions with low resistivities.
However, for DME and THF, which are solvents with small relative dielectric constants, if the alkyl group of the ammonium salt is ethyl, the solubility of these electrolyte salts in DME and THF solvents is very small, and it is very difficult to dissolve them, and the resistivity of the solution cannot be measured. However, when the alkyl group of the ammonium salt is butyl, the solubility of the ammonium salt in DME and THF solvents is greatly increased, and the resistivity of the solution can also be measured.
Reference
[2] H. O. House, E. Feng and N. P. Peet, J. Org. Chem. 36, 2371 (1971).
[3] K. Rousseau, G. C. Farrington and D. Dolphin, ibid. 37, 3968 (1972).
Part 4: Solvent donating and accepting properties and solvent classification
The electron (pair) donor and acceptor properties of a solvent, together with the relative permittivity are factors that affect many reactions and equilibrium that occur in solution. In particular, when comparing the solute behavior of various solvents with high relative permittivity used in the electrode reaction, the effects of the differences in the donor and acceptor properties of the solvents are more pronounced than those in the relative permittivity.
As a measure of the strength of solvent donating and accepting, the donor number (Donor number, expressed in DN) and acceptor number (Acceptor number, expressed in AN) advocated by Gutmann are widely used[4][5].

The method of measuring the number of donors is that in the dichloroethane of the weak donor, the sample solvent (donor) (Body) and SbCl5 (strong acceptor, stronger Lewis acid) occur as shown in equation (6). The heat of the product formed during the addition reaction is the number of donors. The larger the DN value, the stronger the donating property of the solvent.


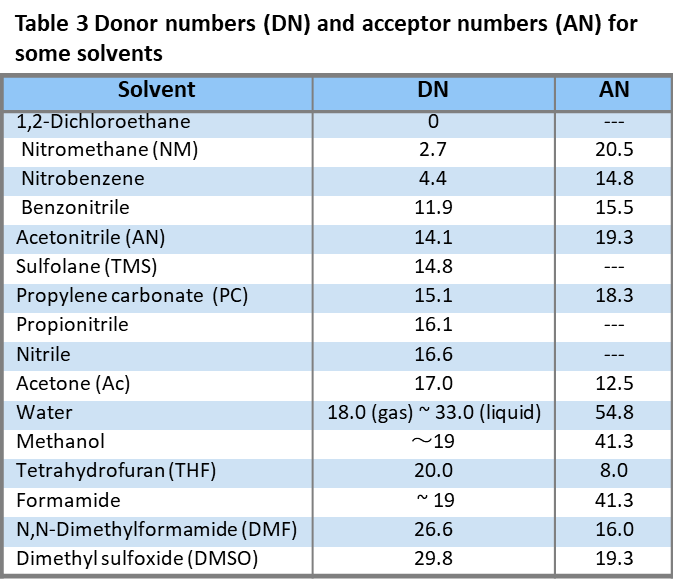
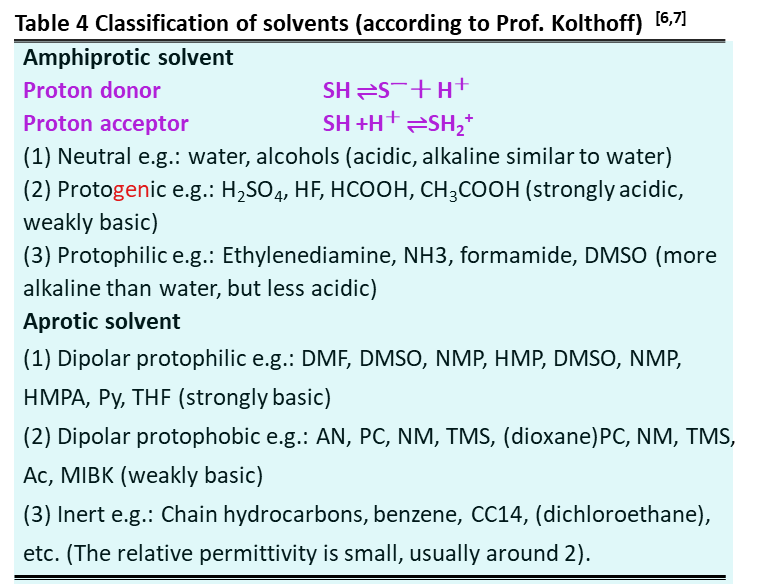
Solvents are usually classified in terms of Bronsted acids and bases, i.e., loss or gain of protons. An example of Prof. Kolthoff's classification of solvents is shown in Table 4. Amphoteric solvents are solvent molecules that are both acidic and basic. When the solvent is denoted by SH, the solvent molecule can release protons and can also receive protons in this way. Among the amphoteric solvents, those with acidic and basic strengths similar to those of water are neutral solvents.
Solvents that are much more acidic than water and much weaker in alkalinity, such as sulfuric acid, hydrofluoric acid, formic acid, acetic acid, etc., are proton donating solvents. On the other hand, solvents that are weaker than water in acidity and much stronger in alkalinity, such as ethylenediamine, NH3, formamide, etc., are protophilic solvents.
On the other hand, aprotic solvents generally have only hydrogen atoms bonded to carbon atoms, so they are weak in releasing protons and donating hydrogen bonds. However, there are strong and weak points in alkalinity. Strong bases are aprotic and weak bases are aprotic. Among aprotic solvents, solvents with large dipole moment and dielectric constant are polar aprotic solvents (dipolar aprotic solvents) and are usually used for electrode reactions.
This is because protons and hydrogen bonding are hardly involved in the reaction in these solvents, which makes possible many phenomena that are not possible in proton solvents such as water. Among the polar aprotonic solvents, AN, PC, TMS, NM, etc. are hydrophobic solvents with little affinity for protons, while these solvents of DMF, etc. are protonophilic. Acetonitrile (AN) Propylene Carbonate (PC) Sulfolane (TMS) Nitromethane (NM).
In reactions where the basicity (electron donating) of the solvent plays an important role, these two groups of solvents exhibit distinctly different properties, and thus need to be appropriately selected according to the purpose.
Reference
[4] V. Gutmann, "The Donor-Acceptor Approach to Molecular Interactions", (1978), Plenum Press.
[5] V. Gutmann, Electrochim. Acta, 21, 661 (1976).
[6] I. M. Kolthoff, Anal. Chem., 46, 1992 (1974).
[7] I. M. Kolthoff and P. J. Elving, ed., "Treatise on Analytical Chemistry", 2nd ed., Part I, Volume 2 (1979).
Part 5: Factors involved in solvation of ions (1)
The solvation of ions involves a variety of factors that are interrelated in the ion solvent interaction.
These factors include:
1) Electrostatic interactions expressed by Brown's equation
2) Donor-acceptor interactions
3) Interaction with anion through hydrogen bonding provided by solvent
4) Interactions of hard and soft acid-base
5) Interaction of electrons from d10 cation giving back to solvent molecule
6) Interactions due to structure formation and structure destruction of solvent
The energy from electrostatic interactions accounts for a significant portion of the total solvation energy, but other factors tend to be more influential when comparing the solvation energies of commonly used solvents with high relative dielectric constants.
a) The solvation of cations is generally closely related to the donor DN (alkalinity) of the solvent
Fig. 5-1 The free energy of K+ ion transfer from acetonitrile to other solvents (ΔGt0(K+)AN → S) (○), and variation of hydrogen electrode potential (●) as a function of the solvent donor number DN [8][9].
Usually the solvation of cations is closely related to the donor (alkalinity) of the solvent, and solvation tends to be stronger in solvents with higher DN. For example, Fig. 2 shows the free energy (ΔGt0(K+)AN → S)(○) of K+ ion transfer from acetonitrile to other solvents as a function of solvent donor number DN, and the hydrogen electrode potentials (which have a linear relationship with the solvation energies of the H+ ions)(●) in various solvents as a function of solvent donor number DN.
It can be seen that there is a nearly linear relationship between the solvation energy of K+ ions and the hydrogen electrode potential (which is linearly related to the solvation energy of H+ ions) (●) and the solvent donor number DN.
b) Ion solvation is closely related to the acceptor property (acidity) of the solvent.
Fig. 5-2 The solvation energy of Cl- Cl- ions transferred from acetonitrile solvent to other non-aprotic solvents (ΔGt0(Cl-)→ S) linearly changes with the acceptor number AN[8][9 ]
The solvation effect of anions is closely related to the acceptability (i.e. acidity) of the solvent. The greater the acceptor number AN of the solvent, the stronger the solvation effect on the anions. In general, small anions such as fluoride, chloride, and hydroxide ions, as well as anions such as acetate, whose negative charge is concentrated on the smaller oxygen atom, have strong hydrogen bond acceptability, so they form in water or alcohols. It has a strong solvation effect. However, these ions have weak solvation in non-aprotic solvents that cannot provide hydrogen bonds and are in a rather high reactive state.
For some large anions, such as iodide ions and perchlorate ions, on the one hand, their solubility in water and alcohol solvents becomes weak because of their weak ability to accept hydrogen bonds, but on the other hand, due to the The stronger the polarity, the stronger the dispersion force will be with non-aprotic solvents of similar polarity. This is why the solvation energies of perchlorate ions in water and alcohols are not much different from those in non-aprotic solvents.
Reference:
[8] V.Gutmann,“The Donor-Acceptor Approach to Molecular Interactions”, (1978),Plenum Press.
[9] U.Mayer,Monatsh. Chem.108,1479 (1977).
Part 6: Factors involved in solvation of ions (2)
(c) The structure of water molecules that can form hydrogen bonds between them, so their entropy value is low.
The structure of water molecules can form hydrogen bonds between them, so its entropy value is low. When hydrophilic ions (many inorganic ions are hydrophilic) enter the water, the strong interaction between the water molecules and the ions causes the overall structure of the water around the ions to be disrupted, increasing their entropy.
However, when hydrophobic ions such as tetraalkylammonium ions (NR4+) and tetraphenylborate ions (Ph4B-) enter water, the water molecules around the ions, which are distant from the ions, further bind and increase their hydrogen-bonded structure (structure formation), which decreases their entropy.
As a result, these hydrophobic macro ions become unstable in water, while they can be more stable in organic solvents without hydrogen bonding structures (see Table 5).
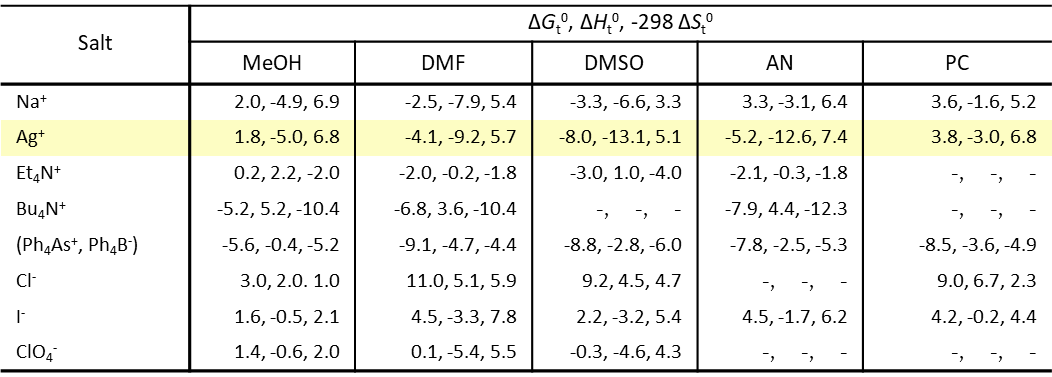
Tetrabutylammonium perchlorate and tetrahexylammonium perchlorate, which are almost insoluble in water, can be well dissolved in organic solvents and used as supporting electrolytes.
(d) When considering Lewis acid-base interactions, it is necessary to distinguish between the "hard" and "soft" properties of acids and bases.
In other words, it is easy for hard acids and hard bases, soft acids and soft bases to interact with each other. This effect can be significant even in the solvation of metal ions.
For example, Fig. 6-1 shows the coordination of the hard base N-methylpyrrolidone (NMP, whose oxygen atom is coordinated with the metal ion) and the soft base N-methylthiopyrrolidone (NMTP, whose S atom is coordinated with the metal ion). Since these half-wave potentials are based on the half-wave potential of the bisbiphenylchromium(I) complex (which is considered to show an almost constant value regardless of the solvent), the metal ions in the two solvents, the solvation effect is that hard acids such as Na+ and K+ have a strong solvation effect in hard alkaline NMP, while soft acids such as Cu+ and Ag+ have a higher degree of solvation in soft alkaline NMTP solvents. Similar phenomena were observed in dimethylformamide DMF and dimethylthioformamide DMTF.
(e) The DN value of acetonitrile (AN) is relatively small, so the solvation of metal ions in AN is generally weak.
However, monovalent metal ions such as Ag+ and Cu+ are different in acetonitrile (AN) solvents and have very strong solvation and stability. (Compare Ag+ Δt0 in acetonitrile and propylene carbon PCs in Table 5). This is due to the d electrons feeding back from the metal ions to the nitrile group. Therefore, silver and monovalent copper ions are not easily reduced to metals in acetonitrile solvents.
Part 7: Acid-base equilibrium and pH range in organic solvents (1)
The reaction of dissociation of HA-type acid in solvent is shown in equation (8). It can be divided into two stages.

The equilibrium constant of the ionization reaction process in the first step is represented by KI, and the equilibrium constant of the dissociation process in the second step is represented by KD (Equation 9). The ionization equilibrium constant Ka obtained by the potentiometric method or conductivity method can be expressed by Equation 10.


Therefore, in order to make Ka larger, both KI and KD need to be larger. This means that the two processes of acid HA ionization and (ion pair) dissociation must occur easily.
During the ionization process, the solvent presses
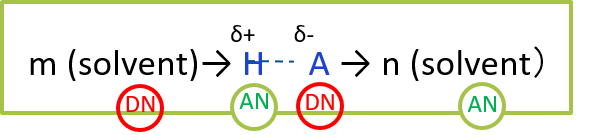
The sequence acts as a donor and an acceptor, and the H-A bond breaks into an ion pair of solvated H+ , and solvated A- . This reaction process is more likely to occur if that H-A bond itself is more easily broken, or if the solvent is more strongly solvated with the H+ and A- ions.
In addition, from the previously mentioned Fuoss equation (3), it can be seen that the larger the relative permittivity of the solvent and the larger the closest distance between the H+ and A- ions, the easier the dissociation of the solvated ion pairs (H+, A- ) will be.

For acids of type HA, in addition to the dissociation reaction, such homoconjugation reactions can occur, and the values of the homoconjugation reaction constants, KfHA2- are shown in parentheses in Table 6.
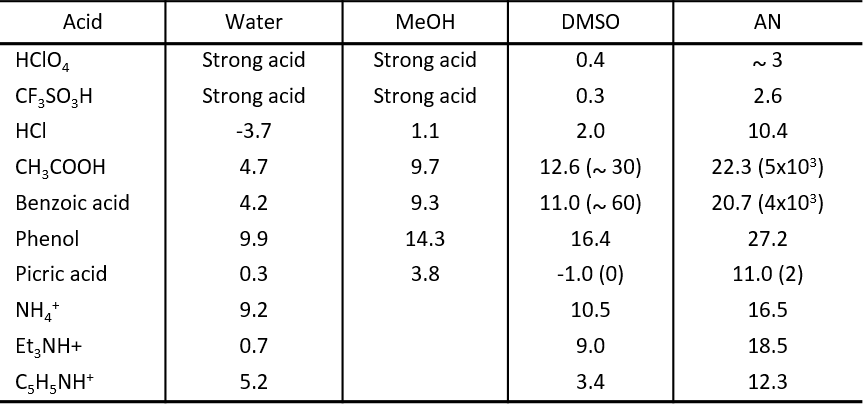
Water, with its relatively strong donor and acceptor properties and high relative dielectric constant, is a solvent that easily dissociates acids.
On the other hand, in acetonitrile solvents, where both donor and acceptor properties are weak, solvation of H+ is difficult, and solvation of A- ions is often difficult as well. As a result, the pKa of the acid is very large, and there is almost no acid that can be completely dissociated. However, in DMSO, which is a strong donor, H+ is strongly solvated, so the pKa is smaller than that in acetonitrile, and acids such as picric acid, which solvate anions relatively easily, can be completely dissociated.
Table 6 shows the pKa for the dissociation of BH+ ⇔ B + H+ for acids of the BH+ type, which is affected by the strength of solvation of BH+, B, and H+, with the effect of solvation of H+ being relatively large. Thus, the pKa in acetonitrile (AN) is much larger than in DMSO. pKa in DMSO is usually approximately the same as in aqueous solutions.
Reference:
[10] I. M. Kolthoff and P. J. Elving,Treatise on Analytical Chemistry, 2nd Ed. Part I, Vol. 2 (1979).
Part 8: Acid-base equilibrium and pH range in organic solvents (2)
In polar organic solvents, as in aqueous solutions, pH buffer solutions can be made by mixing a weak acid HA with its conjugate base A- (using tetraalkylammonium salts with high solubility) or a weak base B with its conjugate acid BH+ (e.g., by using perchlorate).
Dissociation of the BH+ type is usually simple, as shown in the above equation, so the relationship between the composition and buffering capacity of BH+ / B buffer solutions in polar organic solvents is similar to that in aqueous solutions.
However, in HA-A- type buffer solutions, HA and A- can react in a homogeneous conjugation reaction to form HA2-
Conjugation reaction: HA + A- ⇆ HA2-
Conjugation reaction constant: KfHA2- = [HA2-] / ([HA] [A-])
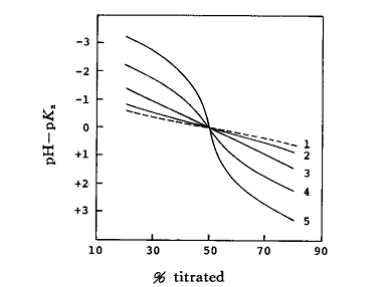
Fig. 8-1 Effect of homogeneous conjugation reaction on the titration curve of HA acid titrated with Et4NOH))
CHAKf HA2- : 1) 0 (in the absense of homogeneous conjugation); 2) 1; 3) 10; 4) 102; 5) 103
The effect of the homogeneous conjugation reaction on the titration curve for the titration of an acid of type HA with tetraethylammonium hydroxide (Et4NOH) is shown in Fig. 8-1 The titration curve without the effect of the homogeneous conjugation reaction is a straight line, and as the conjugation reaction constant Kf increases, the titration curve deviates from the straight line to a greater extent.
The relationship between the composition and buffering capacity of HA-A-type buffer solutions is complex, with homogenization conjugation reactions occurring when both HA and A- are less soluble and more reactive.
In the previous Table 4, the solvents were classified into two main groups: amphoteric and nonprotonic. If we denote amphoteric solvents by SH, the self-ionization reactions occurring in them can be expressed as follows.
Similar to the self-ionization reaction constant for water Kw = [H3O+][OH-], the ease with which the reaction can be carried out can be expressed in terms of the self-ionization constant KSH, i.e., the size of the product of the concentrations of the two ions.
For nonprotonic solvents, it is generally very difficult to obtain the chemical composition corresponding to the S-ion due to the very weak protonation, and even if weak self-ionization can occur, the KSH value obtained will often be less accurate. The self-ionization constant of water at 25 degrees Celsius, i.e., the ion product of water, is 10-14, and the range of pH values that can be used for measurement is roughly 0 - 14. Similarly, based on the KSH values obtained above, the pH ranges that can be measured by various solvents are shown in Fig. 8-2.
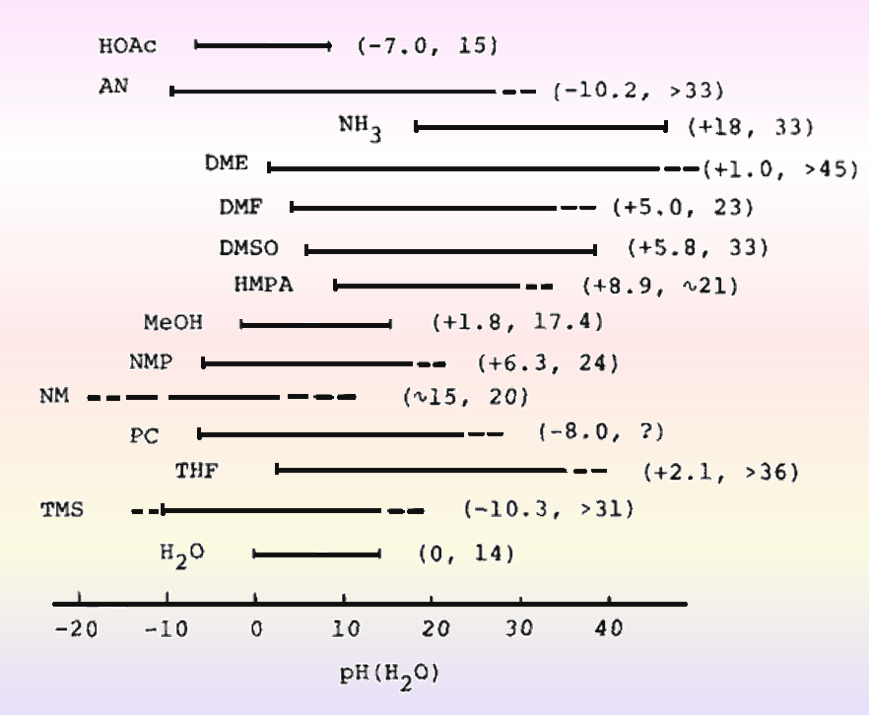
Fig. 8-2 pH range in various solvents (based on water values).
Expressed within ( ) is -logrt(H +W → SH, pKSH)
The pH scales are all based on aqueous solutions, and the minimum pH in each solvent is equivalent to -logγt(H+)w→sH when expressed in terms of the activity coefficient γt(H+)w→sH for the transfer from water to organic solvents.
The smaller the solvent's donor number and the weaker its basicity, the wider its acidic region will be. The smaller the number of acceptors of a solvent, the weaker its acidity, and the wider its basicity region will be. Non-protonic solvents that are sparsely protonated can be utilized because they have a wider acidic and basic range than water.
Stronger acids in solvents with a wide range of acidity, or stronger bases in solvents with a wide range of alkalinity, can be left unreacted with the solvent and remain present in their original state. This can be used for the differential quantification of mixtures of strong acids and bases in non-aqueous solvent titrations, but when appropriate solvents are used in the electrode reaction, strongly acidic (acceptor) and strongly basic (donor) substances that are intermediates or products can be stabilized, which is one of the important reasons for the use of organic solvents in electrode reactions.
Part 9: Supporting Electrolytes
As an electrolyte for electrochemical measurements it should fulfill the following requirements:
1) It should have considerable solubility in the solvent so that the electrolyte is sufficiently conductive.
2) And should have a wide range of measurable potentials.
3) It must also meet the conditions that it does not react with the solvent or various substances involved in the electrode reaction, or that it does not produce unfavorable effects such as alteration of the double layer.
Therefore, the types of support electrolytes that can be used in organic solvents are quite limited compared to those used in aqueous solutions.
For details of the supporting electrolytes used in various solvents, please refer to the literature [1, 11].
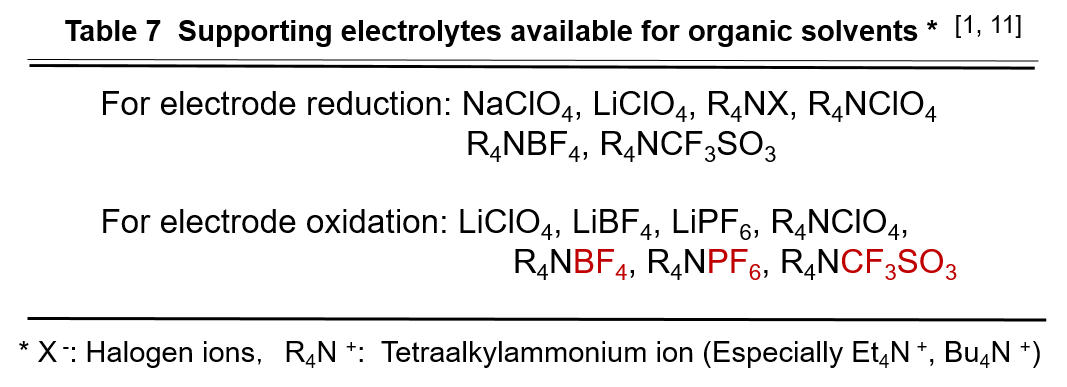
The supporting electrolytes shown in Table 7 can be used in a variety of organic solvents. Of these, the most widely used are the perchlorates of tetraalkylammonium. In particular, tetraethylammonium perchlorate and tetrabutylammonium perchlorate are cheap and readily available because they dissociate readily into ions, have a wide range of measurable potentials for both mercury and solid electrodes, and have little adverse effect on the electrode response.
However, perchlorate has a risk of explosion when dry. Care must be taken when using them. In recent years, the use of tetrafluoroborate (BF4-) and hexafluorophosphate (PF6-) salts, trifluoromethanesulfonates, has increased in lieu of perchlorates, especially in studies of oxidation reactions, and these salts have proved to be superior to perchlorates.
In low dielectric constant solvents such as DME and THF, electrolytes are difficult to dissolve and dissociate into ions, so there are even fewer types of electrolytes to work with, but even in these solvents these electrolytes with large ionic radii of the anion and cation dissolve relatively well and can provide considerable electrical conductivity (see Table 2).
Reference:
[11] Tetsuro Cho, Fundamentals of Electrode Reactions, Kosuke Izutsu, Non-Aqueous Solvents, p. 31; Masamichi Fujihira, Protonated Addition Reactions, p. 109; Tetsuro Cho, Support for Electrolytes, p. 177 (1973), Kyoritsu Press (in Japanese).
Part 10: Potential range of electrochemical measurement (Part 1)
The potential range that can be used for electrode reaction measurements (the range of potentials in which a residual current can be obtained in a solution in which the supporting electrolyte has been dissolved) depends greatly on the solvents and supporting electrolytes that make up the electrolyte, as well as the type of electrodes used for the measurements and the condition of their surfaces. In the following, the potential limits in the direction of the negative potential are discussed first, using mercury and platinum electrodes as examples.
For mercury electrodes: Due to the high overpotential of hydrogen at the mercury electrode, except for acidic solvents, the cations that normally support the electrolyte are reduced before the solvent. Since the tetraalkylammonium ion can be reduced to the amalgam R4N (Hg)n and stabilized, it is suitable as a supporting electrolyte for the reduction reaction. This amalgam can exist relatively stably as a film on the electrode surface in aprotic solvents such as acetonitrile and dimethylformamide, but it reacts immediately with water in aqueous solution. Since alkali metal ions can form stable metal amalgams with mercury. Reduction can occur at a positive potential of 0.5 to 1 V above the tetraalkylammonium ion. In mercury electrodes, the negative potential limit does not change much even with impurity water molecules.
For platinum electrodes: In protonated solvents, e.g. in the presence of water, the negative potential limit is the potential at which hydrogen is produced by reduction of water. However, in aprotic solvents, the reduction of cations in the supporting electrolyte tends to be the negative potential limit. The reduction potential of alkali metal ions can vary considerably depending on the strength of their solubility and may be more positive or more negative than that of the tetraalkylammonium cation. Alkali metal ions are reduced in many nonprotonic solvents and deposited on the electrodes, but when Na+, Li+, etc., are used as cations supporting the electrolyte in a strongly donor HMPA, blue dissolved electrons from the surface of the electrodes enter the solution and become the limit of the negative potential direction.
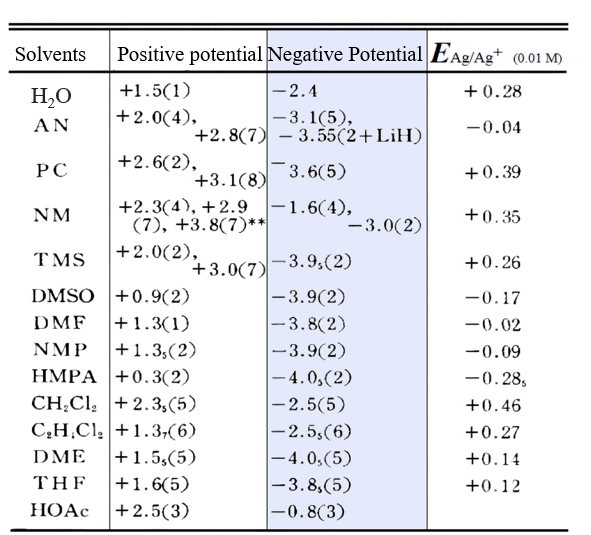
Note: The supporting electrolyte type at a current density of 10µA/mm2 is shown in parentheses.
1. HClO4; 2. LiClO4; 3. NaClO4; 4. Et4NClO4; 5. Bu4NClO4; 6. Hep4NClO4; 7. Et4NBF4; 8. KPF6
The blue background portion of Table 8 shows the limits of the measurable negative potential of the platinum electrode in various solvents. It can be seen that in many cases the range of negative potentials for the platinum electrode is wider than for the mercury electrode.
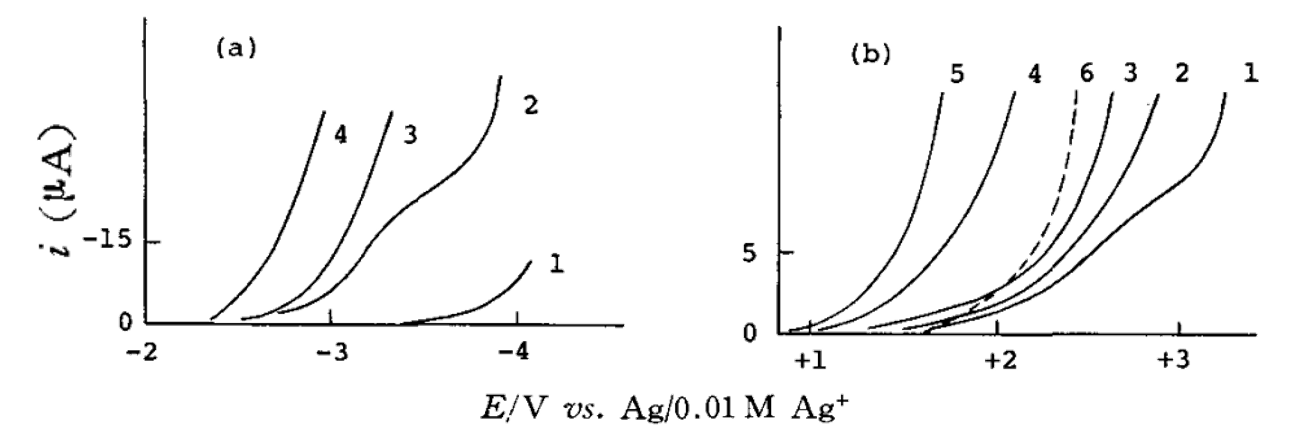
Fig. 10 Effect of water content on residual current on platinum electrode in PC solvent.[12]
(a) Negative potential direction in 0.1mmol/L Bu4NClO4. The concentration of water: 1. ⁓0 M; 2. 0.01 M; 3. 0.03 M; 4. 0.1 M
(b) Positive potential direction in 0.1 M KPF6. The concentration of water: 1. 0.01 M; 2. 0.03 M; 3. 0.1 M; 4. 0.5 M; 5. 1 M (6 is in 0.1M LiClO4 with a water concentration of 0.001 M).
Reference:
[1] A. J. Bard, ed., Electroanalytical Chemistry, Vol. 8, D. Bauer and M. Breant, Solute behavior in solvents and melts, a study by use of transfer activity coefficients, p. 281, Marcel Dekker (1975).
[12] J. Courtot-Coupez and M. L’Her, Bull. Soc. Chim. Fr.,1631 (1970).
Part 11: Potential range of electrochemical measurement (Part 2)
The limits of the positive potential direction are discussed below.
For mercury electrodes, the limit of its positive potential is the oxidation dissolution potential of mercury. When using perchlorate as the supporting electrolyte, the positive potential limit is 0 to +0.4 V. In the presence of halogen ions, the oxidation dissolution potential of mercury will shift in the negative direction, especially in aprotic solvents where anionic solvation is difficult to occur, this negative shift of the positive potential limit will be more obvious.
For mercury electrodes: Due to the high overpotential of hydrogen at the mercury electrode, except for acidic solvents, usually the cations supporting the electrolyte will be reduced before the solvent.. Since the tetraalkylammonium ion can be reduced to the amalgam R4N (Hg)n and stabilized, it is suitable as a supporting electrolyte for the reduction reaction. This amalgam can exist relatively stably as a film on the electrode surface in aprotic solvents such as acetonitrile and dimethylformamide, but it reacts immediately with water in aqueous solution. Since alkali metal ions can form stable metal amalgams with mercury. Reduction can occur at a positive potential of 0.5 to 1 V above the tetraalkylammonium ion. In mercury electrodes, the negative potential limit does not change much even with impurity water molecules.
For platinum electrodes, it can be seen from Table 8 that if perchlorate (R4N+, Li+ or Na+ salts) is used as the supporting electrolyte, the oxidation of the solvent itself becomes the limit in the direction of the positive potential in strong feedstock solvents such as DMSO, DMF, etc., while if the solvent is a weak feedstock solvent, such as acetonitrile, etc., the oxidation potential is limited by the perchlorate ion. As can be seen from the table, the tetrafluoroborate (BF4-) and hexafluorophosphate (PF6-) salts are more suitable for the measurement of oxidation reactions than perchlorate.
For example, in the nitromethane solvent NM the positive potential limit of the perchlorate ion is +2.3 V, whereas for the BF4- ion it is +2.9 V (compared to Fc/Fc+).
In proton sparing non proton solvents (e.g., acrylates PC, etc.), the total potential range can be measured up to about 6 - 7 V. In aqueous solutions, even when measured with mercury electrodes in the negative potential direction and platinum electrodes in the positive potential direction, the total potential range can be measured up to less than 5 V. Therefore, organic solvents are advantageous from the point of view of the potential range.
Therefore, organic solvents are advantageous from the point of view of the potential range, and various electrode reactions that are not possible in aqueous solutions can be carried out in organic solvents.
Part 12: The effect of solvents on electrode reactions
The properties of the solvent, the properties of the supporting electrolyte, and changes in their behavior can have various effects on the electrode reaction.
Let’s first look at the effect of the solvent on the electrode reaction.
• Effects on the electrode reactions of metal ions and complexes
1) The potential of metal ions to be reduced to metal (or metal amalgam) will move towards the negative potential direction with the enhancement of the solvation of metal ions in the solvent, and there is a thermodynamic correspondence between the negative shift of this potential and the solvation energy. The effect of solvents on polarographic half-wave potentials is often used as a means of comparing the dissolution energy of metal ions.
2)Since the decomposition of solvents is not easy to occur in aprotic solvents, those alkaline earth metals, aluminum, zirconium and other ions that do not get a good polarogram due to hydrolysis in aqueous solutions can also obtain better reduction waves.
Reactive metals that react with water, such as alkali metals, can also be precipitated on the electrode in an aprotic solvent.
3) Low-valence metal ion complexes produced by electrode reactions can be stable in nonprotonic solvents.[13][14]
For example, the bipyridyl ligand ion of divalent iron, [Fe(bipy)3]2+, undergoes a 2-electron reduction in aqueous solution to produce a zero-valent iron complex in one step, however, in nonprotonic solvents it undergoes a 1-electron, 3-step, reversible reduction step to produce the more stable [Fe(bipy)3]- as below.
In aqueous solution:

In nonprotonic solvents:

The same is true for various complexes such as Cr, Ni, and Ru bipyridine complexes.
This is because the aprotic solvent is weakly acidic and has difficulty reacting with the ligand (base) of the complex. From the perspective of obtaining potential reference standards that are independent of solvents, these reaction systems deserve everyone's attention.
In addition, since those complexes and organometallic compounds that are insoluble in water can usually be dissolved in organic solvents, these electrode reactions are studied in detail.
• Effect on the electrode reaction of organic compounds
Many organic compounds (R) are reduced by 2 electrons in aqueous solution to generate the hydrogenation reduction product RH2 in one step (Eq. 11).
However, in aprotic solvents, the mechanism of the reduction reaction is divided into two one-electron reduction steps. First, one electron is reduced to generate a fairly stable anionic radical, and then one electron is further reduced to R2- anion.
This phenomenon is very important in the history of the application of organic solvents to electrode reactions and has been extensively studied. Through research, it was found that as the number of solvent acceptors increases, the half-wave potential of anionic radicals generated by reduction in the first step will move forward linearly.
Aoyagi et al. used platinum electrodes in a DME solution of 0.4M tetrabutylammonium perchlorate to achieve four 1-electron reversible oxidation-reduction steps of cyclodecacyclic olefin molecules. And a relatively stable active anion equivalent to R4- was obtained in DME.
In addition, in polar aprotic solvents such as acetonitrile (AN), propylene carbonate (PC), nitromethane (NM), sulfolane (TMS), etc., it can be measured by using appropriate supporting electrolytes. To quite high positive potentials, even the oxidation of benzene, which is difficult to oxidize, can be observed.
The oxidation of organic compounds often generates in the first step a cationic radical (R ⇄ R+ + e), which is usually more unstable than the corresponding anionic radical. In protic solvents and aqueous solutions, these cationic radicals react with the solvent. However, if it is in an aprotic solvent, because there will be no reaction between the solvent and the cationic free radicals, free radical polymerization can occur.
Therefore, when measuring oxidation reactions, it is necessary to use a solvent with appropriate donor (basic) strength to achieve the target reaction.[15]
Reference
[13] T. Saji and S. Aoyagui, Inorg.Nucl. Chem. Lett., 2, 359 (1966).
[14] T. Saji and S. Aoyagui,Electrochim. Acta, 13, 335 (1968).
[15] T. Saji and S. Aoyagui, J. Electroanal. Chem., 102, 139 (1979).
Part 13: Effect of supporting electrolytes
Since the cations and anions supporting the electrolyte react with species or products of opposite polarity, this can have various effects on the electrode reaction.
1) The reduction potential of a metal ion becomes more negative when the metal ion forms an ion pair or complex with the anion of the electrolyte salt.
2) The greater the cationic radius of the electrolyte salt, the easier it is to reduce the metal ion in the more strongly donor solvent (the reduction potential becomes more positive).
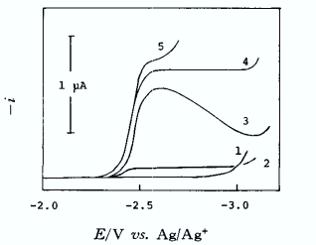
1, Me4N+, Et4 N+; 2, Bu4N+; 3, Hex4N+; 4, Hep4N+; 5, Li+ (0.05 M ClO4- salt each).
Na+ concentration was 0.5 mM.
When the supporting electrolyte cation is tetramethylammonium root, or tetraethylammonium root ion, the sodium ion is not reduced at all, and only a residual current is seen; when the supporting electrolyte cation is tetrabutylammonium root ion a very small reduction wave is obtained, which has the same characteristics as the reaction current.
When the radius of the electrolyte cation becomes further larger, as in the case of lithium ions, tetraheptylammonium root ions, etc., the reduction waveform of Na+ ions is close to a reversible diffusion-controlled waveform.
This phenomenon can be explained qualitatively as follows:
At negative potentials, cations with small radii are preferentially attracted closer to the electrode surface, whereas larger cations are prevented from approaching the surface by the presence of smaller cations. As the electrolyte cations become slightly larger, such as the tetrabutylammonium root ion, this effect becomes less pronounced, and some Na+ is reduced (by osmosis or desolvation) close to the electrode surface. When the cation becomes further larger, there is almost no interference effect and a diffusion-controlled rate reduction wave is obtained.
It can be seen that the effect of the cations of the supporting electrolyte on the reduction of metal ions at negative potentials is very significant in the most feeder (alkaline) HMPA, a phenomenon commonly seen in the reduction reactions of alkali and alkaline-earth metal ions in solvents such as DMSO, DMA, DMF, etc., which are highly feeder.
However, this phenomenon is less pronounced in weak donor solvents such as acetonitrile (AN) or propylene carbonate (PC).
3) Effect of cations supporting electrolytes on the reduction of organic compounds
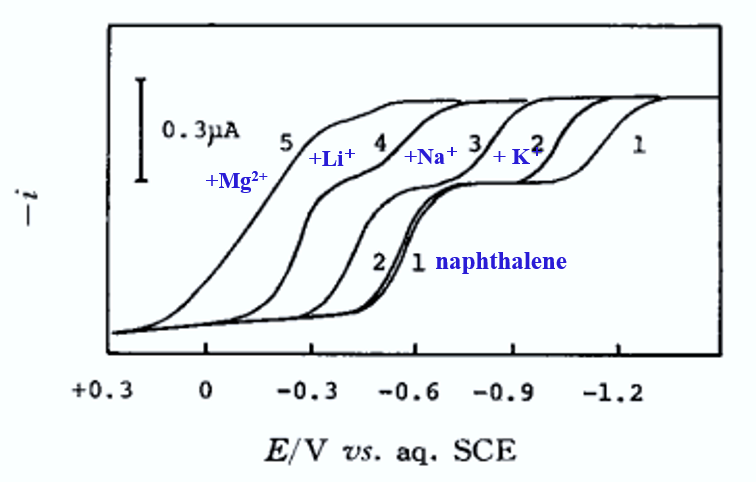
(1): 0.05 M Et4NClO4, 0.5 mM naphthoquinone, (2): (1) ten 5 mM K+, (3): (1) ten 5 mM Na+, (4): (1) ten 5 mM Li+, (5): (1) ten 5 mM Mg2+
In nonprotonic solvents, the anionic radicals of the first-step reduction products of the reduction reactions of organic compounds have the property of forming ion-pairs readily with various metal ions, so that metal salts can be used as the supporting electrolytes, or metal ions can be added to the 4-alkylammonium salt solution during the measurement of the polarograms, and, as shown in Fig. 9, the first reduction wave shifts to a positive potential (from the positive shift of the potential of this half-wave, the ion-pair generation constant can be derived).
It can be observed that the smaller the radius of the metal ion becomes, or the higher the charge number, or the smaller the donor solvent such as acetonitrile AN, or propylene carbonate (PC), the more likely it is that the half-wave potential will shift positively.
We know that the formation of such ion pairs promotes disproportionation and polymerization reactions of R∸ anion radicals (R∸). Therefore, sodium and lithium ion salts are not suitable as support electrolytes for measuring the reduction reactions of organic compounds.
Reactions at the second step where the anionic radical gains a further electron to generate a 2-valent alkyl anion will be more susceptible to supporting electrolyte cations than the first step reactions. The reason for the apparent positive reduction potential in the presence of alkali metal ions can be attributed to the fact that the 2-valent alkyl anion is more susceptible to ion-pair formation with the supporting electrolyte cation than the anion radical. On the contrary, when the radius of the 4-alkylammonium ions increases, the reduction potential gradually shifts toward the negative potential, and the reason for this seems to be that the effect due to the change in the structure of the double electric layer should be considered.
Reference
[17] K. Izutsu, S. Sakura and and T. Fujinaga, Bull. Chem. Soc., 45, 445 (1972); 46, 493, 2148 (1973).
[18] T. Fujinaga, K. Izutsu and T. Nomura, J. Electroanal. Chem., 29, 203 (1971).